- The process of creating a drug - May 23, 2023
- How do scientists model mental disorders in animals? - May 23, 2023
Abstract
Alpha-Bromovalerophenone, also referred to as bromovalerophenone, is an aromatic haloketone in which a benzene ring is substituted with both a pentanoyl group and a bromine atom. This compound plays a crucial role as a precursor in the synthesis of α-PVP, a potent stimulant drug. The following article provides an extensive examination of bromovalerophenone, encompassing its physical and chemical properties, pertinent chemical information, diverse synthesis methodologies, concluding remarks, and an extensive bibliography.
General Information About 2-Bromovalerophenone [1-4]
Other synonyms names of 2-Bromovalerophenone are: 2-bromo-1-phenyl-pentan-1-one; 2-Bromo-1-phenylpentan-1-one; 2-Bromovalerophenone; 2-Bromo-1-phenyl-1-pentanone; bromovalerophenone; alpha-bromovalerophenone
IUPAC Name of 2-Bromovalerophenone: 2-bromo-1-phenylpentan-1-one
CAS number is 49851-31-2
Trade name are bromovalerophenone; alpha-bromovalerophenone; 2-Bromovalerophenone
Physico-Chemical Properties of 2-Bromovalerophenone [1-4]
- Molecular Formula C11H13BrO
- Molar Weight 241.12 g/mol
- Boiling point 290.3 °C
- Melting Point 58.78 °C
- Refraction index 1.541
- Density 1.3 g/mL
- Solubility: Chloroform (Slightly), Methanol (Slightly); in water 31.97 mg/L at 25 °C
- Color/Form: Clear Colourless Oil
Structural formula present on Figure 1.
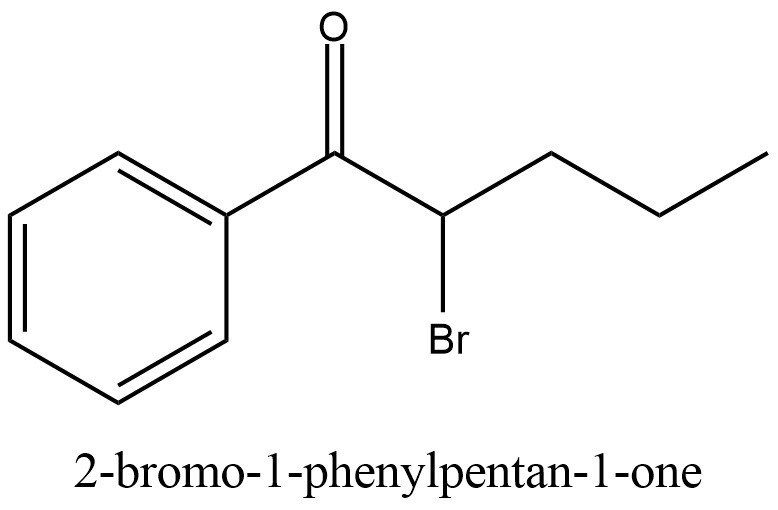
Liquid possible of the 2-Bromovalerophenone can be seen in the picture provided in Figure 2.

Commercial 2-Bromovalerophenone can be seen in the picture provided in Figure 3.
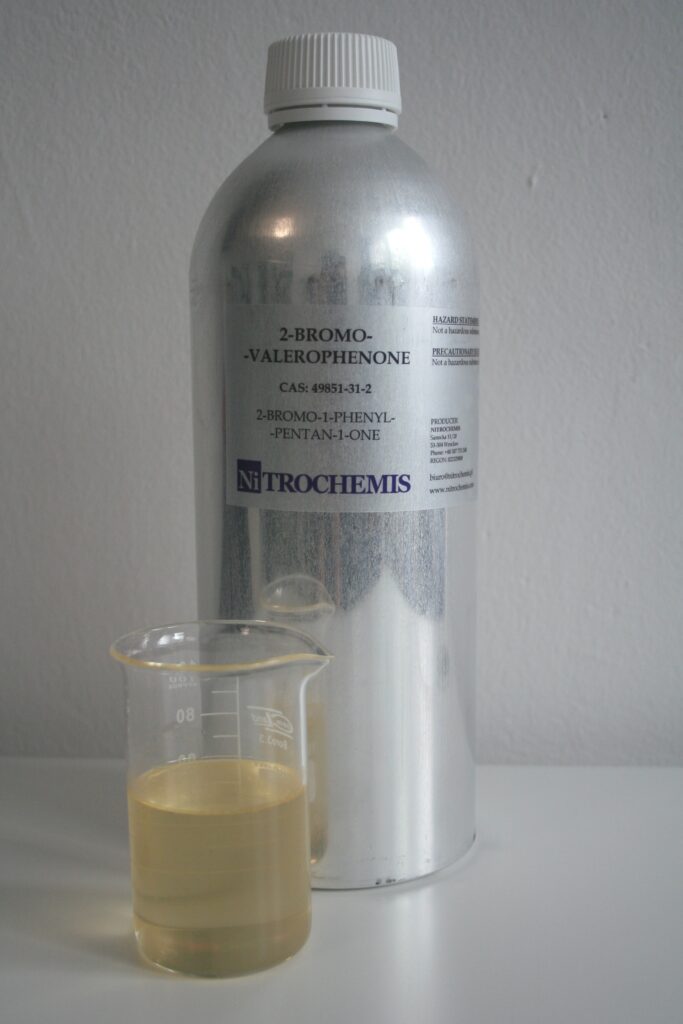
Chemical Information of 2-Bromovalerophenone [5-17]
2-Bromo-1-phenyl-1-pentanone is a unique chemical compound possessing both an aromatic hydrocarbon group, bromine atom and a carbonyl group, rendering it highly reactive. This compound serves as a crucial intermediate in the synthesis of various compounds with diverse applications. Additionally, research has revealed that 2-Bromo-1-phenyl-1-pentanone exhibits notable antimicrobial activity against a wide range of bacteria and fungi. Its ability to combat these microorganisms makes it a promising candidate for pharmaceutical and antimicrobial applications.
Chemical reactions
The Grignard reaction is a pivotal organic chemical process that involves the interaction between a Grignard reagent and a ketone compound. Grignard reagents are organometallic compounds typically synthesized by combining alkyl or aryl halides with magnesium metal in the presence of an ether solvent. When applied to ketones, the Grignard reagent acts as a nucleophile, targeting the electrophilic carbon atom within the ketone’s carbonyl group. This initial step initiates the formation of a carbon-carbon bond connecting the carbon atom from the Grignard reagent with the carbonyl carbon atom of the ketone. Consequently, a new carbon-carbon bond is forged, generating an alkoxide intermediate. However, this alkoxide intermediate is inherently unstable and readily reacts with water or an acidic source, leading to the desired final product. When subjected to hydrolysis with water, the outcome is an alcohol, while an acidic source triggers the formation of the corresponding carboxylic acid. The Grignard reaction with ketones finds extensive application in organic synthesis, facilitating the introduction of new functional groups and the creation of intricate carbon frameworks. It offers a versatile and efficient method for constructing various organic compounds, including alcohols, carboxylic acids, and their derivatives. Due to the sensitivity of Grignard reagents to air and moisture, it is imperative to handle them with utmost care, ensuring that reactions are conducted under anhydrous conditions. Figure 4.
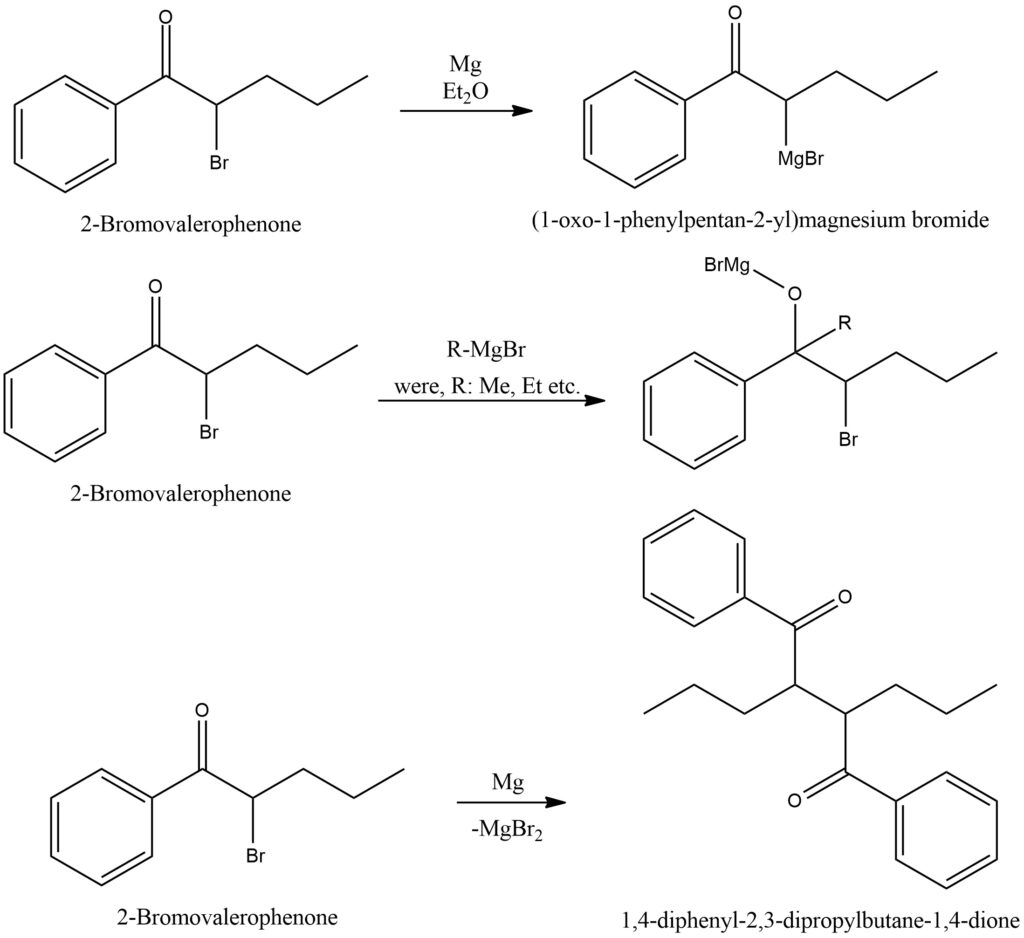
The Darzen condensation, also known as the Darzen glycidic ester condensation, is a chemical transformation that involves the condensation of an α,β-unsaturated acid chloride or anhydride with an alkoxide or phenoxide nucleophile. It was first discovered by the Swiss chemist Victor Darzen in the early 20th century. In the Darzen condensation, the acid chloride or anhydride reacts with the nucleophile, resulting in the formation of a β-hydroxy ester or β-hydroxy ketone. The reaction proceeds by the nucleophile attacking the electrophilic carbonyl carbon of the acid chloride or anhydride, leading to the elimination of a chloride ion or anhydride molecule. This step generates a cyclic intermediate, which undergoes an intramolecular nucleophilic attack by the alkoxide or phenoxide group. As a result, the desired β-hydroxy ester or β-hydroxy ketone is formed. The Darzen condensation is a versatile method for synthesizing β-hydroxy esters and β-hydroxy ketones, which serve as important building blocks in organic synthesis and can be further modified to yield a wide range of functional groups. This reaction can be performed under mild conditions and provides good control over regioselectivity and stereochemistry. Figure 5.

Ketone hydrogenation, also known as ketone reduction, is a chemical process that involves the addition of hydrogen (H2) to a ketone functional group, resulting in the formation of an alcohol. This reaction is extensively utilized in organic synthesis and is commonly catalyzed by transition metal catalysts such as palladium, platinum, or nickel. In ketone hydrogenation, the ketone molecule undergoes reduction in the presence of a hydrogen source and an appropriate catalyst. Molecular hydrogen (H2) gas is often employed as the hydrogen source, and the catalyst facilitates the activation of hydrogen and its addition across the ketone carbonyl group. Consequently, the ketone is converted into an alcohol, incorporating a new hydrogen atom. Ketone hydrogenation is a versatile and valuable transformation in organic chemistry as it enables the selective reduction of ketones to alcohols while preserving the integrity of other functional groups present in the molecule. By adjusting reaction conditions such as temperature, pressure, catalyst choice, and solvent, it is possible to control the selectivity and stereochemistry of the hydrogenation process. The hydrogenation of ketones finds applications in various fields, including pharmaceutical synthesis, the production of fine chemicals, and the derivatization of natural products. It allows for the conversion of carbonyl groups into more versatile and functional alcohol moieties, which can be further manipulated in subsequent chemical transformations. It is important to note that ketone hydrogenation is typically performed under controlled conditions to achieve the desired regioselectivity and avoid over-reduction. The choice of catalyst and reaction parameters should be carefully considered based on the specific ketone substrate and the desired product. Figure 6.
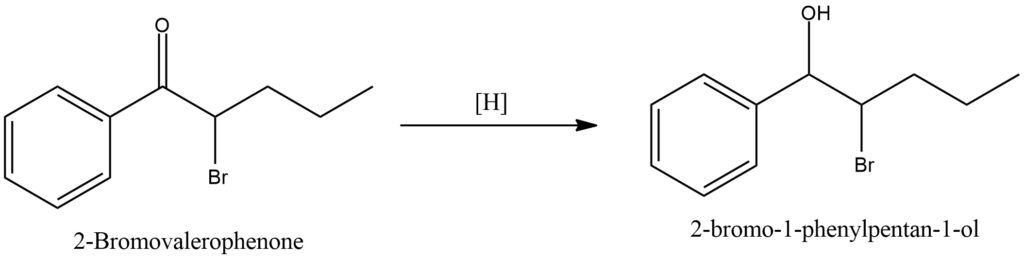
Ketones undergo a reaction with hydroxylamine, leading to the formation of ketoximes while releasing water as a byproduct. This chemical conversion, known as ketoximation, involves the addition of hydroxylamine (NH2OH) to the carbonyl group of the ketone. The addition results in the formation of a C=N bond and converts the carbonyl oxygen into a hydroxyl group. During ketoximation, the nitrogen atom of hydroxylamine, possessing a lone pair of electrons, attacks the electrophilic carbon atom within the ketone’s carbonyl group. This initiates the formation of an intermediate compound, where the oxygen of the carbonyl group forms a bond with the nitrogen atom. Subsequently, water is eliminated from this intermediate, leading to the formation of the ketoxime product. Ketoximes display unique chemical properties due to the presence of the C=N bond. They can serve as versatile intermediates in various organic transformations, including the synthesis of amides, oximes, and other functional groups. These transformations expand the range of chemical modifications and applications of ketoximes in organic chemistry. Figure 7.
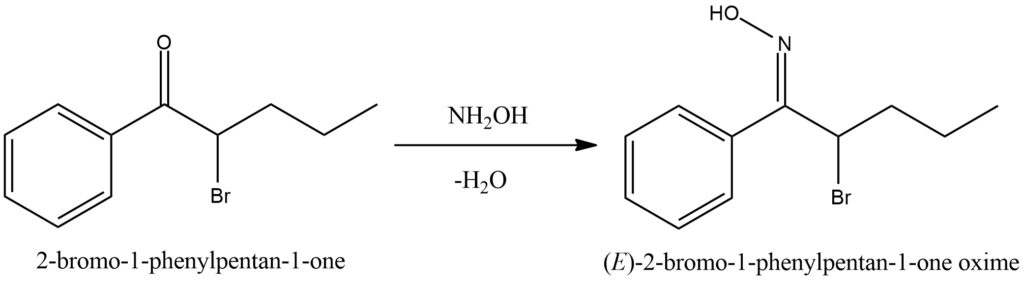
When ketones undergo a reaction with hydrazine, the products formed can be either hydrazones or azines, depending on the reaction conditions. The ratio of hydrazine to ketone plays a crucial role in determining the outcome, where a 1:1 ratio results in the formation of hydrazones, while a 1:2 ratio leads to the formation of azines. In the case of hydrazone formation, one molecule of hydrazine reacts with one molecule of the ketone. The nitrogen atoms of hydrazine act as nucleophiles, attacking the electrophilic carbonyl carbon of the ketone. This results in the formation of a C=N double bond and the conversion of the carbonyl oxygen into a hydrazone group. On the other hand, azine formation occurs when two molecules of hydrazine react with one molecule of the ketone. Both nitrogen atoms of hydrazine participate in the reaction, leading to the formation of a C=N double bond in the ketone and the conversion of the carbonyl oxygen into an azine group. Figure 8.

The Leuckart-Wallach reaction is a chemical transformation that enables the conversion of a carbonyl compound, such as a ketone or aldehyde, into an amine. This reaction is extensively employed in the synthesis of primary and secondary amines. In the Leuckart-Wallach reaction, the carbonyl compound reacts with formic acid (HCOOH) and an amine, typically ammonium formate (NH4HCO2), under elevated temperatures. The reaction proceeds through several sequential steps, starting with the formation of an imine intermediate, followed by subsequent reduction and rearrangement processes. Ultimately, the carbonyl group is converted into an amine group. The specific reaction conditions, including temperature, reaction time, and the choice of catalyst, play a crucial role in determining the selectivity and efficiency of the Leuckart-Wallach reaction. To enhance the reaction rate and yield, suitable catalysts like Raney nickel or platinum on carbon are often employed. They facilitate the transformation by promoting the desired chemical processes. By optimizing these parameters and employing appropriate catalysts, chemists can control the outcome of the Leuckart-Wallach reaction, enabling the synthesis of a wide range of amines for various applications in organic chemistry and pharmaceutical synthesis. Figure 9.
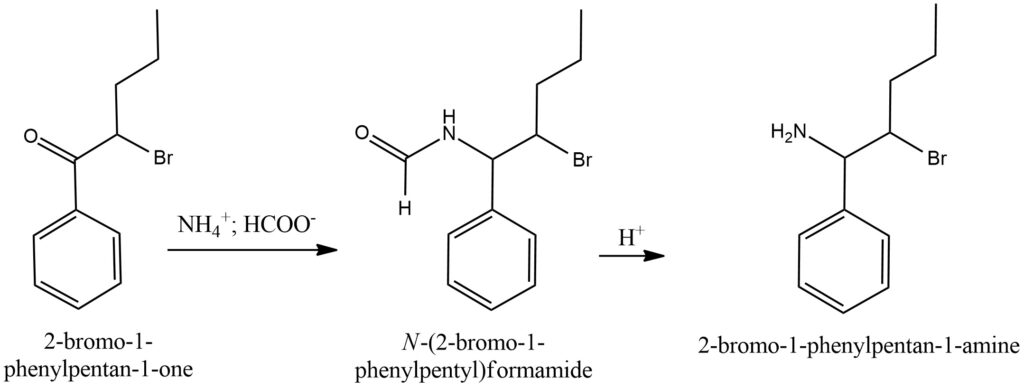
Keto-enol tautomerism is a chemical phenomenon characterized by the interconversion between two constitutional isomers known as the keto and enol forms. This tautomerism occurs in compounds that contain a carbonyl group (keto) and a hydroxyl group attached to an adjacent carbon (enol). The interconversion between the keto and enol forms involves the migration of a proton and the rearrangement of electrons within the molecule. In the keto form, the carbonyl group is predominant, featuring a double bond between the carbon and oxygen atoms. However, under specific conditions such as the presence of a catalyst, heat, or certain solvents, the keto form can undergo a transformation into the enol form. In the enol form, the double bond shifts to the adjacent carbon, resulting in the formation of a hydroxyl group and an alkene bond. The interconversion between the keto and enol forms represents a dynamic equilibrium known as tautomeric equilibrium. The position of the equilibrium is influenced by factors such as temperature, solvent polarity, and the relative stability of each tautomer. In most cases, the keto form is more stable due to the resonance stabilization provided by the carbonyl group. However, in some instances, the enol form may be favored if it exhibits conjugation or intramolecular hydrogen bonding, which enhances its stability. Keto-enol tautomerism has significant implications in organic chemistry as it affects the reactivity, acidity, and overall chemical behavior of compounds. The interconversion between tautomers can influence reaction rates, product formation, and the presence of different functional groups. Understanding and controlling keto-enol tautomerism is crucial for designing and predicting the behavior of molecules in various chemical processes, including drug discovery, natural product synthesis, and material science. Figure 10.
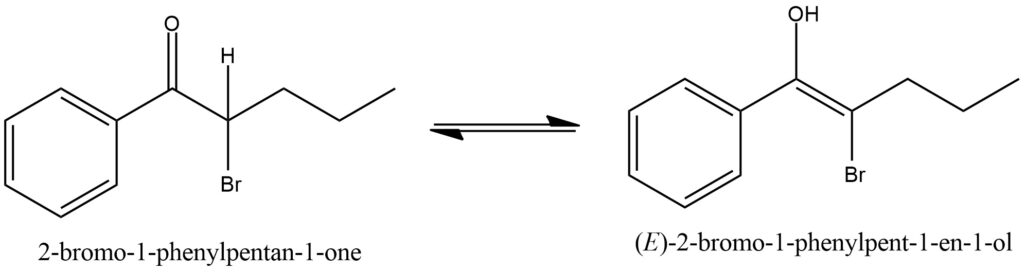
The process of forming imines from ketones is a chemical reaction that involves the interaction between a ketone and a primary amine, resulting in the formation of an imine compound. This reaction is commonly referred to as imine formation or imination. During imine formation, the carbonyl group of the ketone reacts with the amino group (-NH2) of the primary amine. The reaction follows a nucleophilic addition-elimination mechanism. Initially, the lone pair of electrons on the nitrogen atom of the primary amine attacks the electrophilic carbon atom of the ketone’s carbonyl group, leading to the formation of a tetrahedral intermediate. Subsequently, an elimination step occurs, where a water molecule is expelled, leading to the creation of the imine. The imine consists of a carbon-nitrogen double bond (C=N), with the nitrogen atom bonded to an alkyl or aryl group derived from the primary amine. The imine formation reaction can be catalyzed by either acid or base. Acidic conditions generally favor imine formation, while basic conditions promote enamine formation. Enamines involve the addition of a proton to the nitrogen atom of the imine, resulting in the formation of an enamine compound. Figure 11.
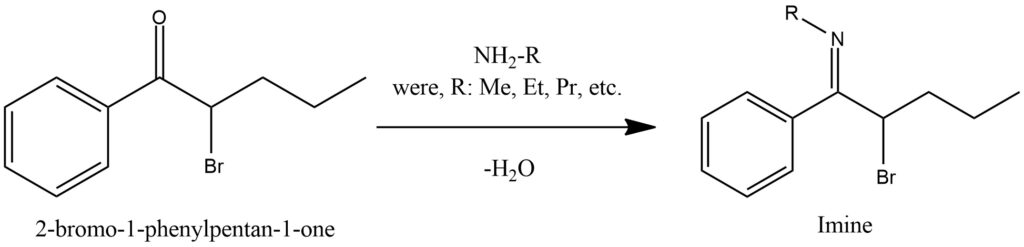
The formation of hemiketals is a chemical process that occurs when an alcohol reacts with a carbonyl compound, resulting in the creation of a hemiketal functional group. Hemiketals are characterized by a carbon-oxygen-carbon (C-O-C) linkage. This reaction typically involves an alcohol molecule reacting with an aldehyde or ketone via a nucleophilic addition-elimination mechanism. Initially, the lone pair of electrons on the oxygen atom of the alcohol attacks the electrophilic carbon atom of the carbonyl group, leading to the formation of a tetrahedral intermediate. Subsequently, an intramolecular proton transfer takes place, leading to the elimination of a water molecule. This process ultimately forms a hemiketal, with the oxygen atom of the alcohol bonded to the carbon atom of the carbonyl group, creating a new C-O-C linkage. The formation of hemiketals is reversible, and the equilibrium position is determined by the relative stability of the hemiketal and the starting materials. Factors such as temperature, concentration, and the presence of catalysts can influence the equilibrium. Hemiketals play a crucial role as intermediates in organic chemistry and find various applications. They can undergo further reactions, including acid-catalyzed cyclization or rearrangement, to generate more complex molecules. In carbohydrate chemistry, hemiketals are commonly involved in the formation of cyclic sugar structures. It is important to note that hemiketals can be converted to stable ketals through subsequent reactions with alcohol molecules. This process involves the formation of a new carbon-oxygen-carbon linkage and serves to protect the carbonyl group. Figure 12.
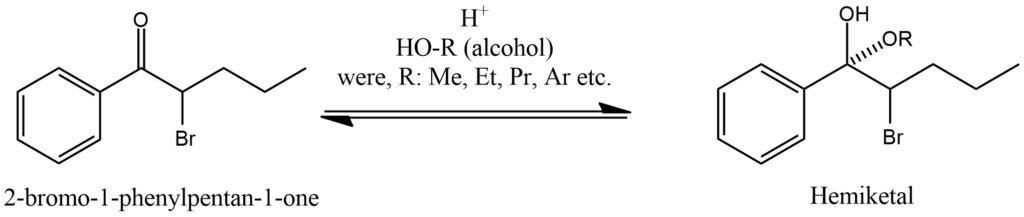
Ketones can be oxidized to form carboxylic acids when treated with strong oxidizing agents. However, compared to aldehydes and primary alcohols, ketones are generally more resistant to oxidation. The oxidation of ketones typically necessitates the use of vigorous conditions, including elevated temperatures and powerful oxidizing agents. During ketone oxidation, the carbon-carbon bond within the ketone undergoes cleavage, resulting in the production of a mixture of carboxylic acids. The carboxylic acids formed have a reduced number of carbon atoms compared to the original ketone because the cleavage of the carbon-carbon bond leads to the loss of one carbon atom. Potassium permanganate (KMnO4), chromic acid (H2CrO4), and Jones reagent (CrO3/H2SO4) are common examples of strong oxidizing agents employed in ketone oxidation. These agents provide the necessary energy to break the carbon-carbon bond and facilitate the conversion of ketones into carboxylic acids. The oxidation of ketones is a significant transformation in organic chemistry and finds applications in various industries. It enables the conversion of ketones into carboxylic acids, which are versatile compounds utilized in pharmaceuticals, polymers, flavors, and other sectors. Figure 13.
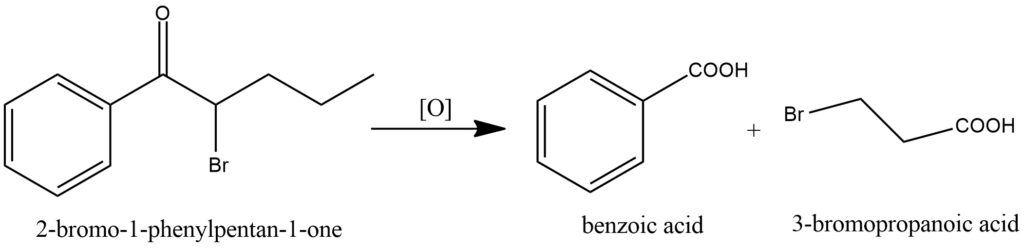
Ketones are capable of undergoing reduction reactions to produce alcohols. One common approach to ketone reduction involves the utilization of metal hydrides, such as sodium borohydride (NaBH4) or lithium aluminum hydride (LiAlH4). These metal hydrides function as reducing agents by donating a hydride ion to the carbonyl carbon of the ketone, resulting in the formation of a metal alkoxide salt. When a ketone is treated with a metal hydride, such as NaBH4, in the presence of a suitable solvent like methanol or ethanol, the carbonyl group selectively reacts with the metal hydride. The hydride ion is added to the carbonyl carbon, leading to the formation of an alkoxide intermediate, which coordinates with the metal cation (e.g., Na+). This intermediate is stabilized through coordination with the metal ion, preventing further reactions and facilitating its isolation. To obtain the alcohol product from the metal alkoxide intermediate, hydrolysis is typically conducted. Hydrolysis involves the addition of water, which results in the cleavage of the metal-alkoxide bond and the formation of the desired alcohol. Hydrolysis can be accomplished by treating the metal alkoxide salt with an acid or simply by adding water. The choice of hydrolysis conditions depends on the specific reaction requirements and desired purity of the product. Another method for ketone reduction involves the use of sodium cyanoborohydride (NaBH3CN). NaBH3CN is a milder reducing agent compared to metal hydrides and is often employed when reaction conditions require greater selectivity. Similar to metal hydrides, NaBH3CN donates a hydride ion to the carbonyl carbon, resulting in the reduction of the ketone to an alcohol. This reagent is particularly useful for reducing ketones in the presence of other functional groups that can also undergo reduction since it exhibits greater tolerance towards these groups. Figure 14.

The alpha-bromination of ketones involves the addition of molecular bromine (Br2) or bromine compounds to the alpha-position of the ketone group, resulting in the formation of alpha-bromoketones. This reaction can be carried out with the assistance of catalytic amounts of other substances, such as acids or Lewis acids, which serve to activate the bromine and impart regioselectivity to the reaction. When a ketone is treated with bromine or a bromine compound in the presence of an appropriate catalyst, the bromine molecule adds to the alpha-carbon atom of the ketone, leading to the formation of an alpha-bromoketone. The catalyst facilitates the activation of the bromine molecule, promoting its interaction with the ketone and directing the addition to the desired alpha-position.Figure 15.

The reaction between 2-bromo-1-phenyl-1-pentanone and hydrochloric acid gives chlorobenzene. Figure 16.
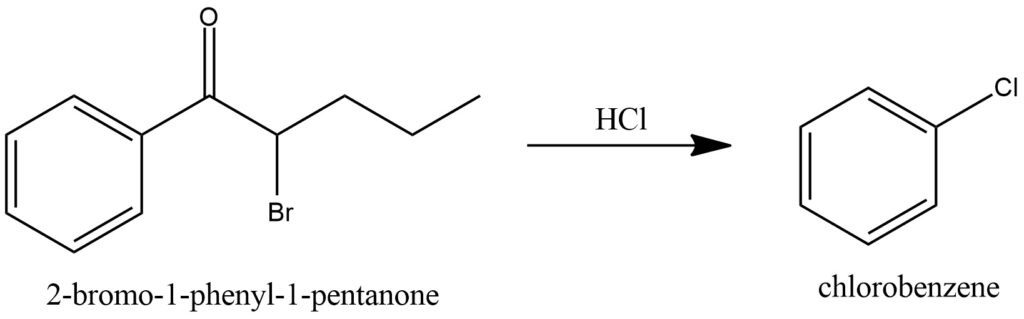
The reaction of 2-Bromovalerophenone with triethylamine hydrochloride (Et3N·HCl) in the presence of acetonitrile (CH3CN) and dichloromethane (CH2Cl2) can yield bromochloropentane. Figure 17.

α-PVP synthesis from 2-Bromovalerophenone. Figure 18.

Synthesis of 2-Bromovalerophenone [18, 19]
The synthesis of 2-Bromovalerophenone is carried out by brominating valerophenone according to the scheme shown in Figure 19.

Conclusion
Bromovalerophenone represents a typical example of aromatic ketones and haloketones, exhibiting the characteristic properties of both ketones and halocompounds. Additionally, bromovalerophenone serves as a valuable precursor for the synthesis of α-PVP (alpha-pyrrolidinopentiophenone). With the application of suitable reaction conditions and reagents, the transformation of bromovalerophenone into α-PVP, a derivative of valerophenone, can be achieved. In essence, bromovalerophenone holds significant importance in organic chemistry, serving as a versatile compound that enables the synthesis of diverse molecules, including α-PVP, through appropriate chemical transformations and reactions. Its utility in various applications underscores its value as a key building block in synthetic endeavors.
Bibliography
- https://pubchem.ncbi.nlm.nih.gov/compound/2-Bromo-1-phenyl-pentan-1-one
- https://www.chemspider.com/Chemical-Structure.8880595.html?rid=11e2e1bc-ce1a-4caf-8610-a7ae1fa2ed2f
- https://www.biosynth.com/p/FB75180/49851-31-2-2-bromo-1-phenyl-1-pentanone
- https://www.trc-canada.com/product-detail/?B689020
- https://en.wikipedia.org/wiki/Darzens_reaction
- https://en.wikipedia.org/wiki/Ketone
- https://en.wikipedia.org/wiki/Imine
- https://en.wikipedia.org/wiki/Hemiacetal
- https://en.wikipedia.org/wiki/Carbonyl_reduction#Aldehyde_and_ketone_reduction
- https://en.wikipedia.org/wiki/%CE%91-Halo_ketone
- https://en.wikipedia.org/wiki/Haloform_reaction
- https://en.wikipedia.org/wiki/Willgerodt_rearrangement
- https://en.wikipedia.org/wiki/Baeyer%E2%80%93Villiger_oxidation
- https://en.wikipedia.org/wiki/Alpha-Pyrrolidinopentiophenone
- https://bbgate.com/media/%CE%B1-pvp-mdpv-synthesis-from-2-bromovalerophenone.47/
- https://bbgate.com/threads/alpha-pvp-synthesis-1-10kg-scale-complete-video-tutorial.12/
- https://en.wikipedia.org/wiki/Pyrrolidine
- https://bbgate.com/threads/alpha-pvp-synthesis-1-10kg-scale-complete-video-tutorial.12/
- https://bbgate.com/media/2-bromovalerophenone-synthesis-from-valerophenone.48/