- The process of creating a drug - May 23, 2023
- How do scientists model mental disorders in animals? - May 23, 2023
Abstract
MDP1P, also known as 3,4-Methylenedioxypropiophenone, is a precursor for the synthesis of Methylone. This article presents a comprehensive overview of MDP1P, including its general information, physico-chemical properties, pharmacology in recreational use, effects and symptoms, hepatotoxicity, use street names, prices and approximate dosage, dangerous interactions, legal status, synthesis of MDP1P , conclusion, and bibliography.
General Information About MDP1P [1-7]
Other synonyms names of MDP1P are: 1-(benzo[d][1,3]dioxol-5-yl)propan-1-one; 1-(1,3-Benzodioxol-5-yl)-1-propanone; 3′,4′-(Methylenedioxy)propiophenone; 1-(1,3-Benzodioxol-5-yl)propan-1-one; 1-Propanone, 1-(1,3-benzodioxol-5-yl)-; 3′,4′-Methylenedioxypropiophenone; 3,4-Methylenedioxypropiophenone; 5-Propanoyl-1,3-benzodioxole; 3,4-Methylenedioxyphenyl ethyl ketone; 3,4-Methylenedioxy Propiophenone
IUPAC Name of MDP1P: 1-(1,3-benzodioxol-5-yl)propan-1-one
CAS number is 28281-49-4
Trade names is MDP1P
Different analogs of MDP1P and MDP2P. This Analogs are presented on Figure 1.
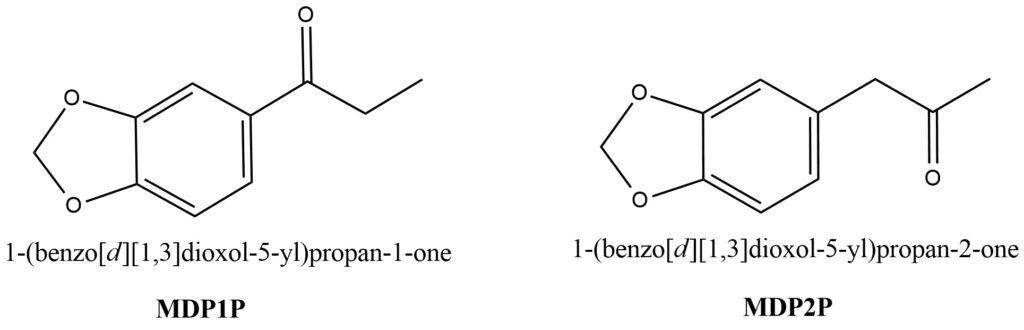
Physico-Chemical Properties of MDP1P [1-7]
- Molecular Formula C10H10O3
- Molar Weight 178.18 g/mol
- Boiling point 165-168 °C (20 mmHg); 312.9286-316.8494 °C (760 mmHg)
- Melting Point 35-39 °C
- Density 1.21 g/mL
- Index of Refraction 1.546
- Solubility: Water Solubility 704.9 mg/L at 25 °C
- Color/Form: solid
Structural formula present on Figure 2.
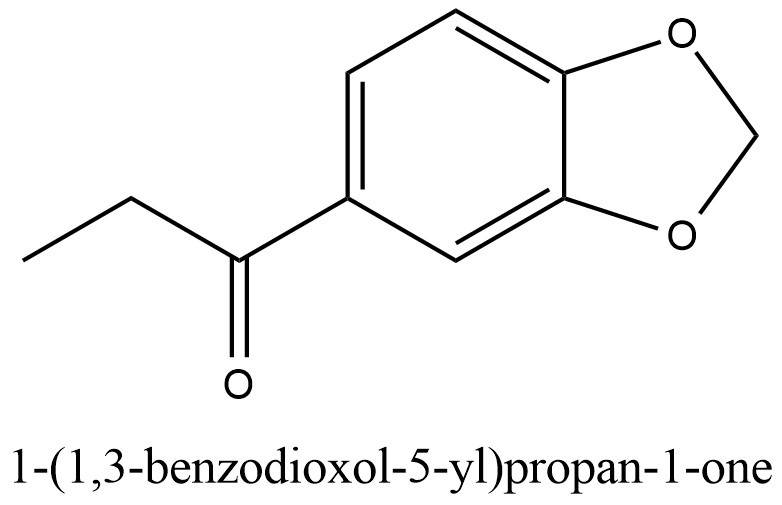
Powder and crystalline solid possible of the MDP1P can be seen in the picture provided in Figure 3.
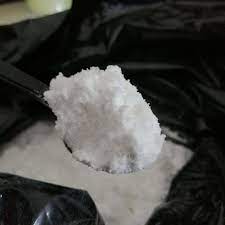
Chemical Information of MDP1P [1-7]
3,4-Methylenedioxypropiophenone (MDP1P) is a synthetic compound with a fatty acid chain that exhibits remarkable properties. It has demonstrated potent activity against leishmania, making it a promising candidate for further research and development. Additionally, MDP1P serves as a crucial intermediate in the synthesis of various drugs.
The synthesis of 3,4-Methylenedioxypropiophenone involves acylation reactions utilizing chloride and acetylcholinesterase inhibition. Its unique molecular docking properties have been extensively analyzed using computational methods like profiling and profile analysis.
Apart from its synthetic origins, 3,4-Methylenedioxypropiophenone is also found naturally in some Piper plant species. Notably, studies have shown that it can either dominate the essential oil of Piper marginatum or be completely absent from it. The chemical’s prevalence varies across different regions, with the highest concentrations observed in Manaus, Melgaço, Belterra, Monte Alegre, and Alta Floresta.
MDP1P plays a pivotal role as a precursor in the synthesis of methylone and various other substituted methylenedioxy-phenethylamine derivatives. One common method of preparation involves the Grignard reaction between ethylmagnesium bromide and piperonylonitrile.
In the United States, MDP1P is not classified as a scheduled drug at the federal level nor listed by the DEA as a controlled substance. However, it’s important to note that “3,4-methylenedioxy-propiophenone,” along with “2-Bromo-3,4-Methylenedioxypropiophenone” and “3,4-methylenedioxy-propiophenone-2-oxime,” are classified as Schedule I controlled substances in the state of Florida, making their purchase, sale, or possession illegal in that jurisdiction.
Chemical reactions
The reaction known as the Grignard reaction is a crucial process in organic chemistry that plays a fundamental role in synthesizing organic compounds. It involves the intriguing interplay between a Grignard reagent and a ketone compound. Grignard reagents, which are organometallic compounds, are typically produced by combining alkyl or aryl halides with magnesium metal in the presence of an ether solvent. This specific combination sets the stage for the Grignard reaction with ketones.
In the context of this reaction, the Grignard reagent acts as a nucleophile, launching an attack on the electrophilic carbon atom residing within the carbonyl group of the ketone. This initial interaction triggers the formation of a carbon-carbon bond, connecting the carbon atom from the Grignard reagent to the carbonyl carbon atom of the ketone. As a result, a fresh carbon-carbon bond is formed, and an intermediate known as an alkoxide is generated.
It is important to note that this alkoxide intermediate is not stable and readily reacts with either water or an acidic source, leading to the desired end product. When treated with water, the Grignard reagent undergoes hydrolysis, resulting in the production of an alcohol. Conversely, if an acidic source is utilized, it drives the formation of the corresponding carboxylic acid. Figure 4.
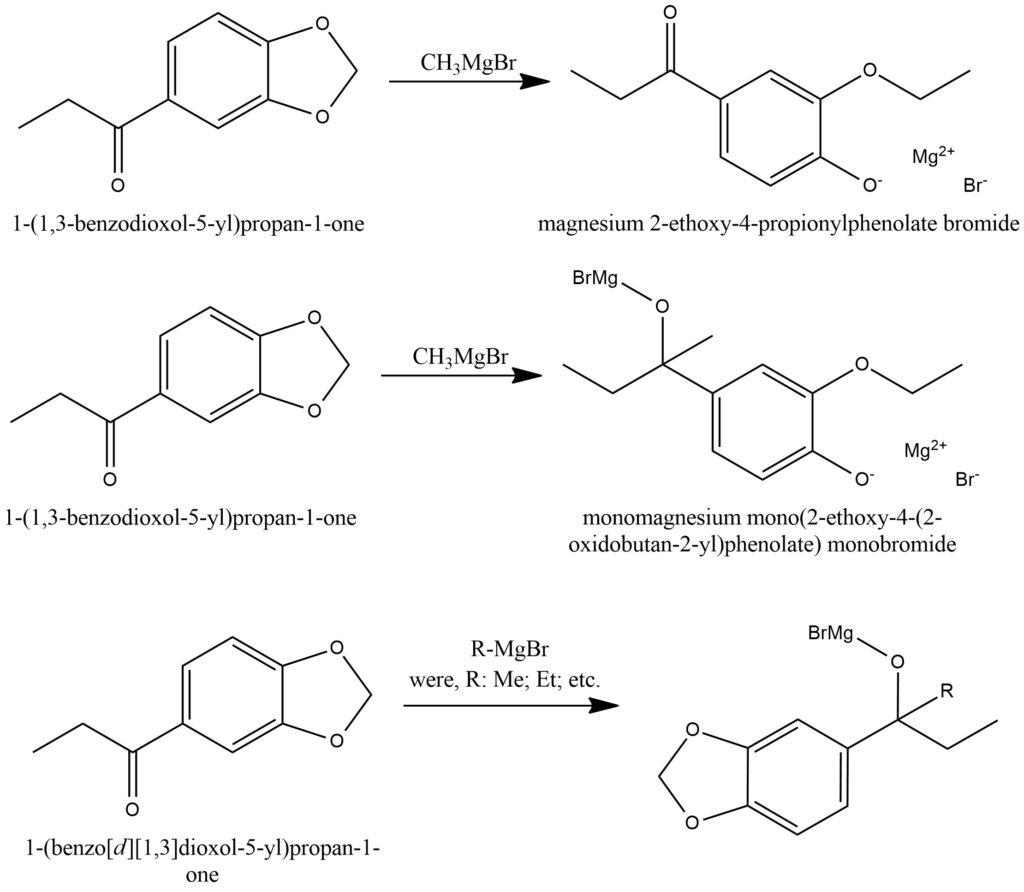
The Darzen condensation, also known as the Darzen glycidic ester condensation, is a chemical transformation that involves the condensation of an α,β-unsaturated ester or ketone with an alcohol or phenol nucleophile. This reaction was initially discovered by the French chemist Victor Darzen in the early 20th century. In the context of the Darzen condensation, the ester or ketone undergoes a reaction with the nucleophile, leading to the formation of a β-hydroxy ester or β-hydroxy ketone. The reaction proceeds by the nucleophile attacking the electrophilic carbon of the ester or ketone, subsequently resulting in the elimination of an alcohol or phenol molecule. This step leads to the formation of a cyclic intermediate, which then undergoes an intramolecular nucleophilic attack by the alcohol or phenol group. As a consequence, the desired β-hydroxy ester or β-hydroxy ketone is formed. The Darzen condensation serves as a versatile method for synthesizing β-hydroxy esters and β-hydroxy ketones, which are essential building blocks in organic synthesis and can be further modified to yield a wide range of functional groups. This reaction can be conducted under mild conditions and provides good control over regioselectivity and stereochemistry. Figure 5.
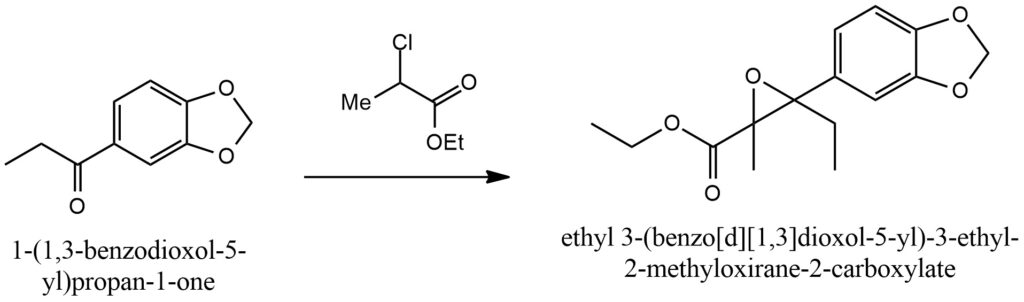
Ketone reduction, also known as ketone hydrogenation, is a fundamental chemical process that involves the reduction of a ketone functional group through the addition of hydrogen (H2), resulting in the formation of an alcohol. This reaction is widely utilized in organic synthesis and is typically catalyzed by transition metal catalysts, such as palladium, platinum, or nickel.
In the context of ketone hydrogenation, a ketone molecule is subjected to reduction in the presence of a suitable catalyst and a hydrogen source. Molecular hydrogen (H2) gas is commonly employed as the hydrogen source, while the catalyst aids in the activation of hydrogen and its subsequent addition across the ketone’s carbonyl group. This transformative step converts the ketone into an alcohol by incorporating a new hydrogen atom.
Ketone hydrogenation is a versatile and valuable transformation in organic chemistry, as it allows for the selective reduction of ketones to alcohols while maintaining the integrity of other functional groups within the molecule. By adjusting various reaction parameters such as temperature, pressure, catalyst choice, and solvent, it is possible to control the selectivity and stereochemistry of the hydrogenation process. Figure 6.
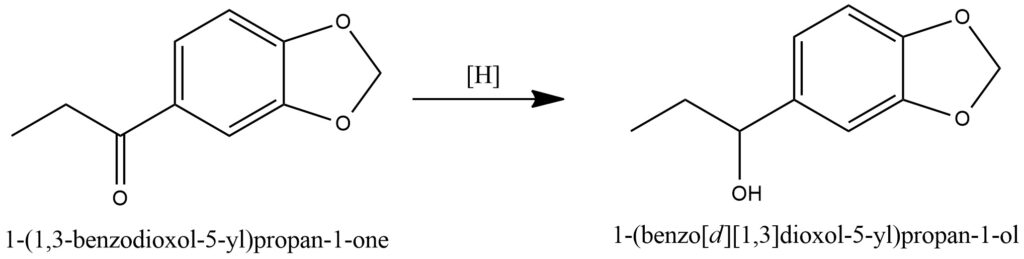
Ketones can undergo a chemical reaction with hydroxylamine, leading to the formation of ketoximes while releasing water as a byproduct. This transformation, known as ketoximation, involves the addition of hydroxylamine (NH2OH) to the carbonyl group of the ketone. The addition of hydroxylamine results in the formation of a C=N bond, converting the carbonyl oxygen into a hydroxyl group.
During the ketoximation process, the nitrogen atom of hydroxylamine, which possesses a lone pair of electrons, attacks the electrophilic carbon atom within the ketone’s carbonyl group. This initiates the formation of an intermediate compound, where the oxygen of the carbonyl group forms a bond with the nitrogen atom. Subsequently, water is eliminated from this intermediate, leading to the formation of the ketoxime product.
Ketoximes exhibit unique chemical properties due to the presence of the C=N bond. They can serve as versatile intermediates in various organic transformations, including the synthesis of amides, oximes, and other functional groups. Their ability to undergo further reactions allows for the incorporation of diverse chemical functionalities into the ketoxime scaffold, enabling the synthesis of a wide range of organic compounds. Figure 7.
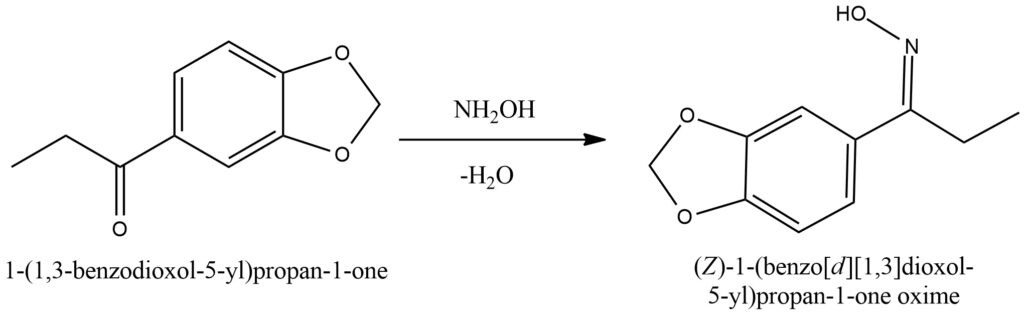
When ketones undergo a reaction with hydrazine, the resulting products can be either hydrazones or azines, depending on the specific reaction conditions. The ratio of hydrazine to ketone plays a crucial role in determining the product outcome. A 1:1 ratio of hydrazine to ketone typically leads to the formation of hydrazones, while a 1:2 ratio favors the formation of azines.
In the case of hydrazone formation, one molecule of hydrazine reacts with one molecule of the ketone. During this process, the nitrogen atoms of the hydrazine act as nucleophiles, attacking the carbonyl carbon of the ketone. This attack results in the formation of a C=N double bond, and the carbonyl oxygen is transformed into a hydrazone group.
On the other hand, azine formation occurs when two molecules of hydrazine react with one molecule of the ketone. In this scenario, both nitrogen atoms of the hydrazine actively participate in the reaction. The nucleophilic attack by the nitrogen atoms leads to the formation of a C=N double bond in the ketone, and the carbonyl oxygen is converted into an azine group.
The formation of hydrazones and azines through ketone-hydrazine reactions provides a valuable means of modifying ketones and introducing different functional groups. The specific product obtained can be controlled by adjusting the ratio of hydrazine to ketone. By leveraging this reaction, chemists can access a diverse array of compounds with varying chemical properties and applications. Figure 8.
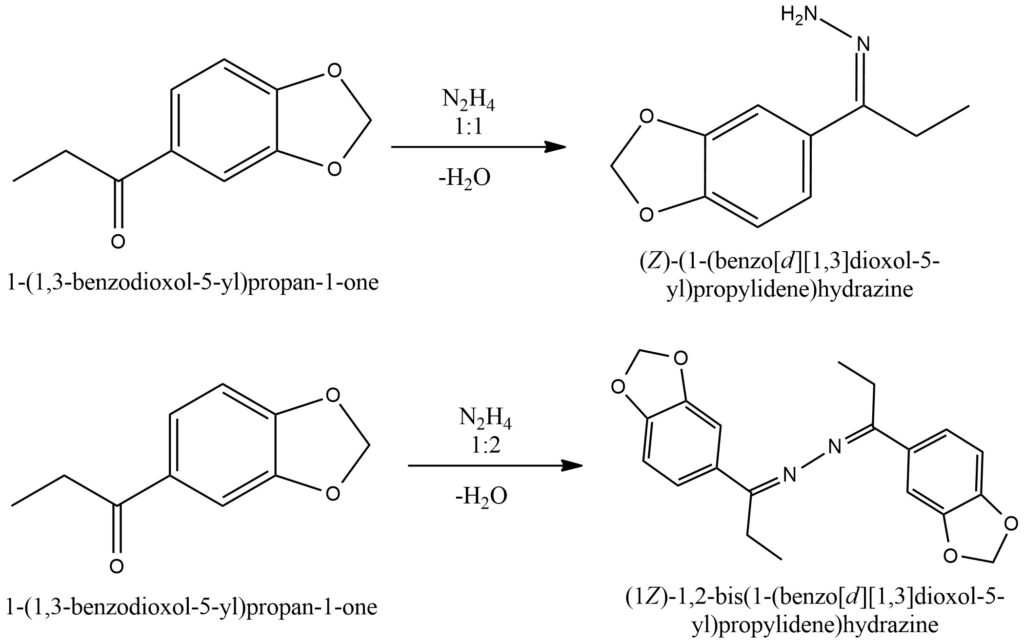
The Leuckart-Wallach reaction is a highly useful chemical transformation that enables the conversion of carbonyl compounds, such as ketones or aldehydes, into amines. This reaction serves as a valuable method for synthesizing primary and secondary amines.
In the Leuckart-Wallach reaction, the carbonyl compound reacts with formic acid (HCOOH) and an amine, typically ammonium formate (NH4HCO2), under elevated temperatures. The reaction proceeds through a series of steps, starting with the formation of an imine intermediate, followed by subsequent reduction and rearrangement processes. Ultimately, the carbonyl group is transformed into an amine group. Figure 9.
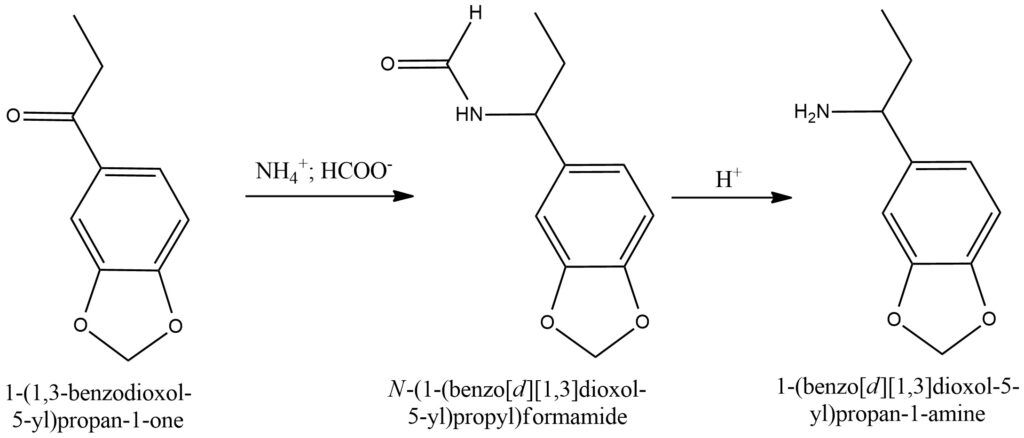
Keto-enol tautomerism is a chemical phenomenon that involves the interconversion between two constitutional isomers, known as the keto and enol forms. This tautomerism occurs in compounds that contain a carbonyl group (keto) and a hydroxyl group attached to an adjacent carbon (enol). The interconversion between the keto and enol forms is driven by the migration of a proton and the rearrangement of electrons within the molecule.
In the keto form, the carbonyl group is predominant, with a double bond between the carbon and oxygen atoms. However, under certain conditions, such as the presence of a catalyst, heat, or specific solvents, the keto form can convert into the enol form. In the enol form, the double bond shifts to the adjacent carbon, forming a hydroxyl group and an alkene bond.
The interconversion between the keto and enol forms is an example of tautomeric equilibrium. The equilibrium position is influenced by factors such as temperature, solvent polarity, and the stability of each tautomer. In most cases, the keto form is more stable due to the resonance stabilization provided by the carbonyl group. However, in some instances, the enol form can be favored if it possesses conjugation or intramolecular hydrogen bonding, which increases its stability. Figure 10.

The process of imine formation from ketones is a chemical reaction that occurs when a ketone reacts with a primary amine, resulting in the creation of an imine. This reaction is commonly referred to as imine formation or imination. During imine formation, the carbonyl group of the ketone reacts with the amino group (-NH2) of the primary amine. The reaction follows a nucleophilic addition-elimination mechanism. Initially, the lone pair of electrons on the nitrogen atom of the primary amine attacks the electrophilic carbon atom of the ketone’s carbonyl group, resulting in the formation of a tetrahedral intermediate. This intermediate compound then undergoes an elimination step, where a water molecule is expelled, leading to the formation of an imine. The imine consists of a carbon-nitrogen double bond (C=N), with the nitrogen atom bonded to an alkyl or aryl group derived from the primary amine.
The imine formation reaction can be catalyzed by either acid or base. Acidic conditions generally promote imine formation, whereas basic conditions tend to facilitate enamine formation. Enamine formation involves the addition of a proton to the nitrogen atom of the imine, resulting in the formation of an enamine.
Imines are highly valuable intermediates in organic synthesis and find diverse applications. They can serve as crucial building blocks for the synthesis of various compounds, including pharmaceuticals, agrochemicals, and dyes. Furthermore, imines exhibit significant biological activities and are utilized as ligands in coordination chemistry. Figure 11.
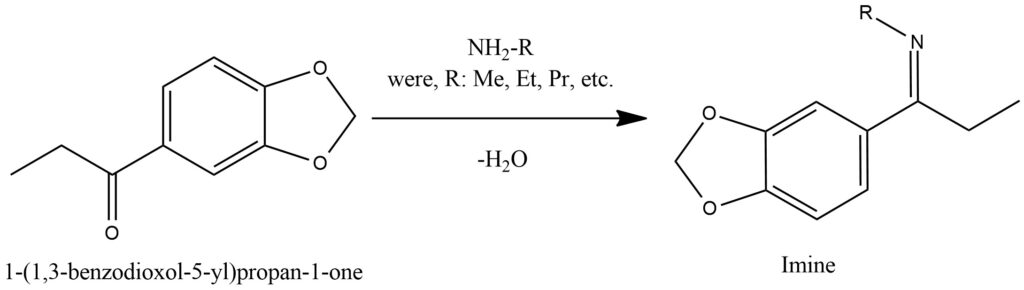
The process of hemiketal formation is a chemical reaction that occurs when an alcohol reacts with a carbonyl compound, leading to the creation of a hemiketal. Hemiketals are functional groups characterized by a carbon-oxygen-carbon (C-O-C) linkage. During the formation of hemiketals, an alcohol molecule reacts with a carbonyl compound, such as an aldehyde or ketone. The reaction follows a nucleophilic addition-elimination mechanism. Initially, the lone pair of electrons on the oxygen atom of the alcohol attacks the electrophilic carbon atom of the carbonyl group, resulting in the formation of a tetrahedral intermediate. Subsequently, an intramolecular proton transfer occurs, leading to the elimination of a water molecule. This process ultimately yields a hemiketal, where the oxygen atom of the alcohol becomes bonded to the carbon atom of the carbonyl group, forming a new C-O-C linkage.
The formation of hemiketals is a reversible process, and the position of the equilibrium depends on the stability of the hemiketal and the starting materials. Various factors, including temperature, concentration, and the presence of catalysts, can influence the equilibrium. Hemiketals play a significant role as intermediates in organic chemistry and find diverse applications. They can undergo further reactions, such as acid-catalyzed cyclization or rearrangement, to form more complex molecules. In carbohydrate chemistry, hemiketals are particularly prevalent, as they are crucial for the formation of cyclic sugar structures. It is important to note that hemiketals can also be converted to stable ketals through subsequent reactions with alcohol molecules. This process involves the formation of a new carbon-oxygen-carbon linkage and serves to protect the carbonyl group. Figure 12.
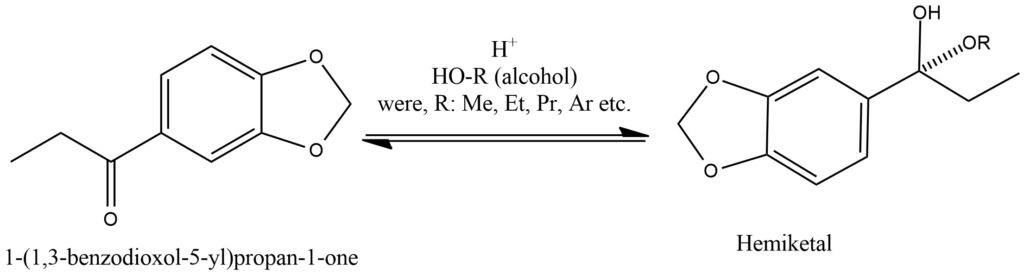
Under the influence of powerful oxidizing agents, ketones can undergo oxidation, leading to the formation of carboxylic acids. However, compared to aldehydes and primary alcohols, ketones are generally more resistant to oxidation. The oxidation of ketones typically necessitates harsh conditions, including elevated temperatures and the use of strong oxidizing agents. During this oxidation process, the carbon-carbon bond within the ketone structure is cleaved, resulting in the formation of a mixture of carboxylic acids. The carboxylic acids generated possess fewer carbon atoms than the original ketone due to the loss of one carbon atom during the cleavage of the carbon-carbon bond. Potassium permanganate (KMnO4), chromic acid (H2CrO4), and Jones reagent (CrO3/H2SO4) are commonly employed strong oxidizing agents for ketone oxidation. These agents supply the necessary energy to break the carbon-carbon bond and facilitate the conversion of ketones into carboxylic acids.
The oxidation of ketones holds significant importance in organic chemistry and finds numerous applications. It enables the transformation of ketones into carboxylic acids, versatile compounds widely used in industries such as pharmaceuticals, polymers, and flavors. The selectivity of the oxidation reaction can be influenced by factors such as the choice of oxidizing agent, reaction conditions, and the presence of other functional groups within the molecule. It is crucial to exercise caution and carefully control the reaction conditions during ketone oxidation due to the hazardous nature of strong oxidizing agents and the potential formation of undesired byproducts. Furthermore, the selection of the oxidizing agent and reaction conditions should be based on the specific ketone substrate and the desired outcome of the reaction. Figure 13.
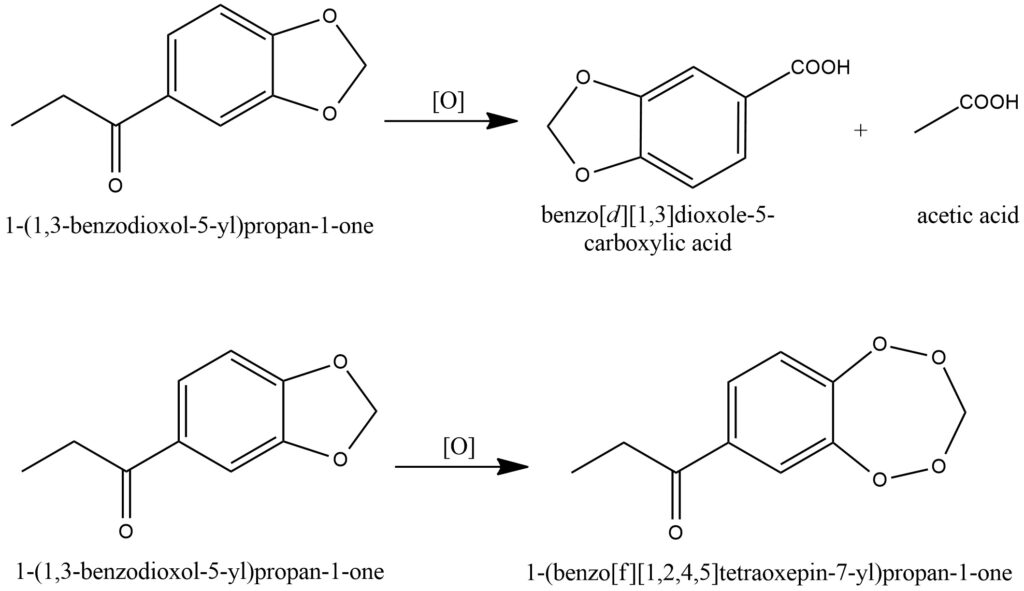
Ketones possess the ability to undergo reduction reactions, leading to the formation of alcohols. A commonly employed method for ketone reduction involves the utilization of metal hydrides, such as sodium borohydride (NaBH4) or lithium aluminum hydride (LiAlH4). These metal hydrides serve as reducing agents by donating a hydride ion to the carbonyl carbon of the ketone, resulting in the formation of a metal alkoxide salt. When a ketone is treated with a metal hydride, such as NaBH4, in the presence of a suitable solvent like methanol or ethanol, the carbonyl group selectively reacts with the metal hydride. The hydride ion adds to the carbonyl carbon, leading to the formation of an alkoxide intermediate, which is coordinated with the metal cation (e.g., Na+). The coordination with the metal ion stabilizes this intermediate, preventing further reactions and facilitating its isolation. To obtain the alcohol product from the metal alkoxide intermediate, hydrolysis is commonly employed. Hydrolysis involves the addition of water, resulting in the cleavage of the metal-alkoxide bond and the formation of the desired alcohol. Hydrolysis can be achieved by treating the metal alkoxide salt with an acid or simply by adding water. The choice of hydrolysis conditions depends on the specific reaction requirements and desired product purity. Another method for ketone reduction involves the use of sodium cyanoborohydride (NaBH3CN). NaBH3CN is a milder reducing agent compared to metal hydrides and is often employed when the reaction conditions require higher selectivity. Similar to metal hydrides, NaBH3CN donates a hydride ion to the carbonyl carbon, resulting in the reduction of the ketone to an alcohol. This reagent is particularly useful for reducing ketones in the presence of other functional groups that are also reducible, as it exhibits greater tolerance towards these groups. Figure 14.
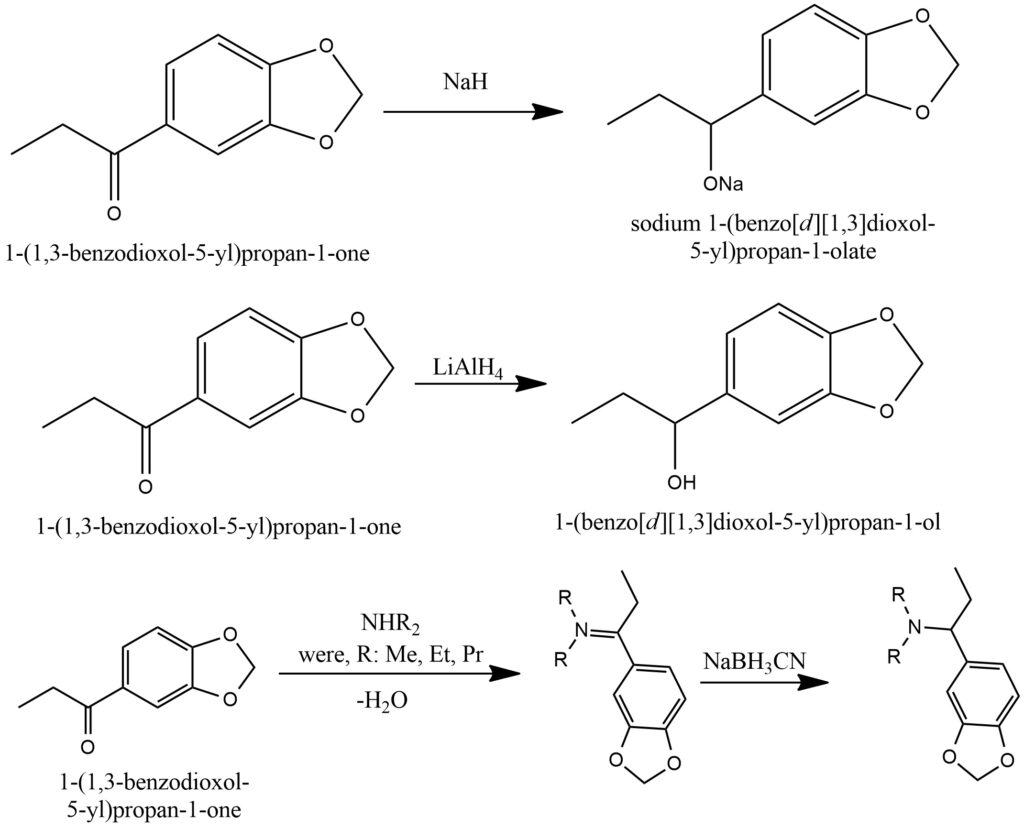
The alpha-bromination of ketones involves the addition of molecular bromine (Br2) or bromine compounds to the alpha-position of the ketone group, resulting in the formation of alpha-bromoketones. This reaction can be conducted in the presence of catalytic amounts of other substances, including acids or Lewis acids, which serve to activate the bromine and enhance the regioselectivity of the reaction. Alpha-bromoketones hold significant importance as intermediates in organic synthesis. They can function as valuable starting materials for various subsequent transformations, such as acylation, aldol condensation, and other reactions that facilitate the creation of complex molecular structures.Figure 15.

The Baeyer-Villiger oxidation is an organic transformation that converts a ketone or cyclic ketone into an ester or lactone, respectively, utilizing peroxyacids or peroxides as oxidizing agents. This reaction is named after Adolf von Baeyer and Victor Villiger, both of whom independently discovered this process. In the Baeyer-Villiger oxidation, the ketone substrate reacts with a peroxyacid or peroxide, such as peracetic acid (CH3CO3H) or hydrogen peroxide (H2O2), in the presence of an acid or base catalyst. The peroxyacid or peroxide acts as an electrophilic oxidizing agent, targeting the carbonyl group of the ketone and forming a cyclic intermediate called a peroxycarboxylic acid ester. Subsequently, this intermediate undergoes rearrangement, with the migration of an alkyl or aryl group, ultimately yielding the desired ester or lactone product. Figure 16.
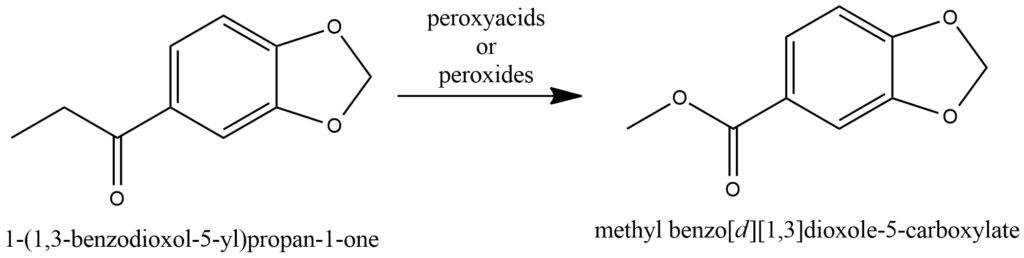
Methylone, also known as 3,4-methylenedioxy-N-methylcathinone (MDMC) synthesis from MDP1P. Figure 17.
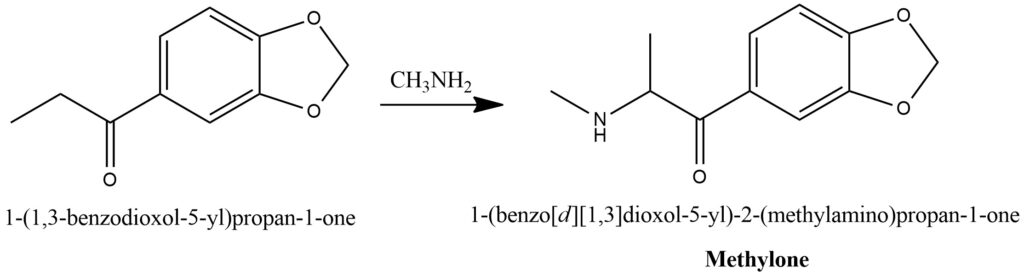
Under the influence of diazoacetic ether, the MDP1P undergoes ring expansion. Figure 18.
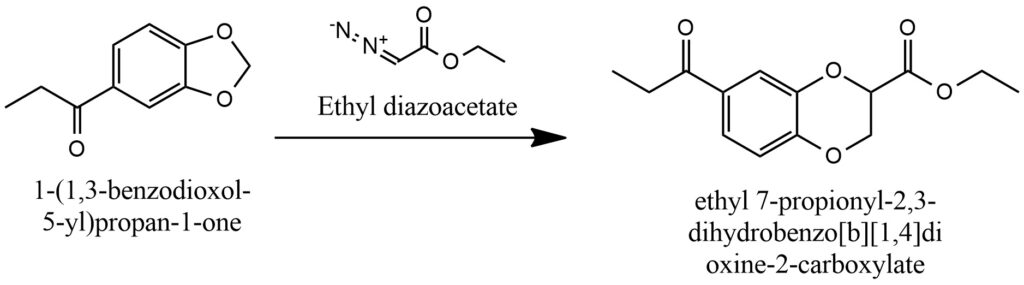
Synthesis of MDP1P [4, 7, 8]
MDP1P serves as a valuable precursor for the synthesis of methylone and a range of other substituted methylenedioxy-phenethylamine derivatives. Its preparation involves a Grignard reaction, wherein ethylmagnesium bromide reacts with piperonylonitrile. The synthesis of MDP1P follows a specific scheme depicted in Figure 19.
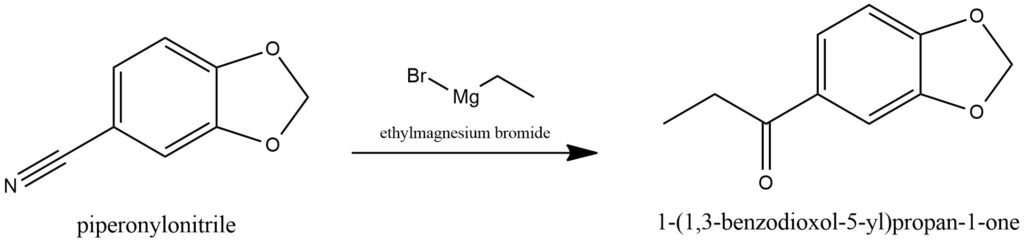
Conclusion
MDP1P is a structural isomer of MDP2P. It is present in certain plants of the Piper genus. It is used in the synthesis of the drug methylenedioxypyrovalerone (MDPV), Methylone and similar compounds.
Bibliography
- https://pubchem.ncbi.nlm.nih.gov/compound/1-_1_3-Benzodioxol-5-yl_-1-propanone
- https://www.molinstincts.com/structure/1-benzo-d-1-3-dioxol-5-yl-propan-1-one-cstr-CT1002701514.html
- http://www.chemspider.com/Chemical-Structure.86372.html
- https://en.wikipedia.org/wiki/3,4-Methylenedioxypropiophenone
- https://www.biosynth.com/p/FM25828/28281-49-4-34-methylenedioxy-propiophenone
- https://www.sigmaaldrich.com/UA/en/product/aldrich/cds001550
- https://en.wikipedia.org/wiki/Methylone
- https://en.wikipedia.org/wiki/Methylenedioxypyrovalerone